From Sonogenetics to Pyramidal Neurons
by Lindsay Borthwick
Research Highlights from Kavli Institutes in Neuroscience
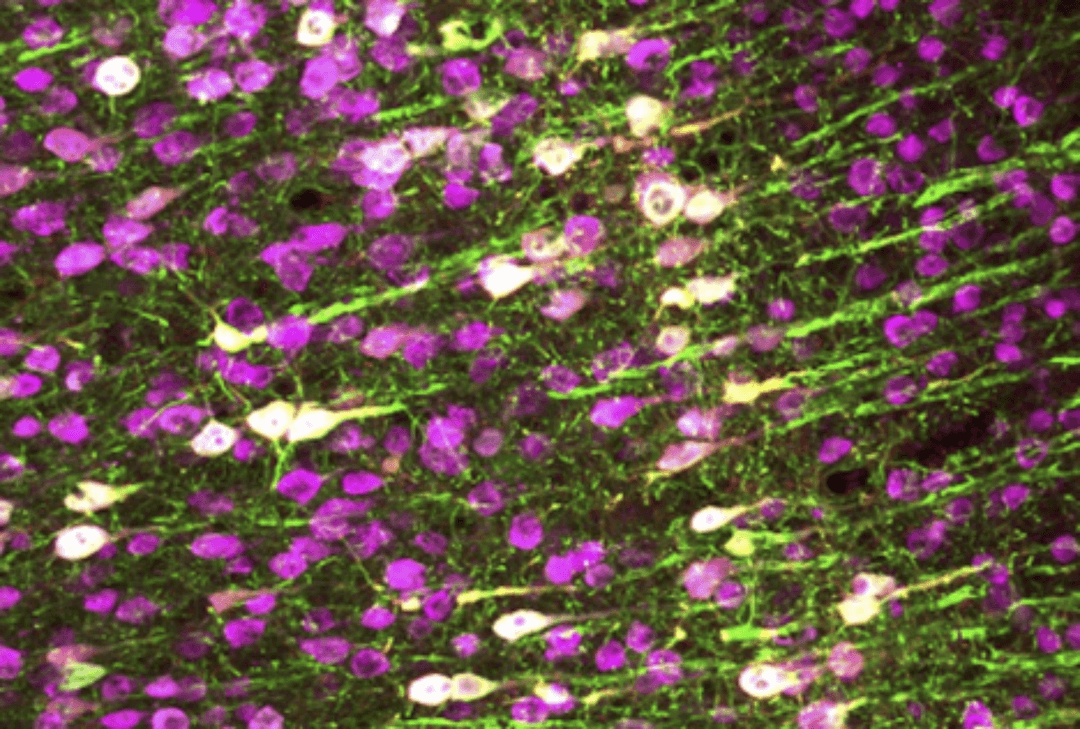
The Author
In this roundup of new research from the Kavli Neuroscience Institutes, we highlight a few discoveries that could eventually have a profound impact on the lives of people living with neurological and psychiatric disorders, starting with a new way to activate nerve cells with sound. The method was developed by researchers affiliated with the Kavli Institute for Brain and Mind in San Diego. Up the coast of California, at the Kavli Institute for Fundamental Neuroscience, researchers have identified the cells and cellular mechanisms that are likely responsible for a rare, debilitating movement disorder. A third team, including researchers at the Kavli Institute for Brain Science in New York City, shed light on why some people with COVID-19 lose their sense of taste and smell.
One day, we may treat the brain with sound
Every now and then, a discovery comes along that has the potential to dramatically change research and treatment for the brain and other organs. One recent example is optogenetics, which can be used to activate cells with light. Now, researchers affiliated with the Kavli Institute for Brain and Mind (KIBM) at the Salk Institute for Biological Studies, have developed a similarly powerful method. Termed “sonogenetics,” it uses ultrasound instead of light to control how a cell behaves. It hinges on the discovery of an ultrasound-sensitive protein that can be engineered into various cell types. Like optogenetics, sonogenetics is non-invasive. But using ultrasound instead of light offers several advantages: high-frequency sound waves can penetrate deeper into tissue; they are also safely and routinely used in healthcare today. If researchers can optimize the new method, it might eventually be used to stimulate the brain in diseases such as epilepsy and Parkinson’s. Neurobiologist Sreekanth Chalasani, who led the research, recently published a report about the technique in the journal Nature Communications. His team used ultrasound to activate human cells in a dish and brain cells in live mice, causing the mice to flex their limb muscles.
In a Q&A in Spectrum, Chalasani describes the twists and turns in the research leading up to his discovery, and the challenge ahead to bring the technique to the clinic: “The real challenge, I think, for both sonogenetics and optogenetics, is how do you deliver these proteins to the part of the brain that you care about? And how would you do it in a child - or in a human? We don’t have an answer for that.”
How COVID-19 interrupts taste and smell
Loss of taste and smell is a common symptom of COVID-19 but one that has puzzled researchers because the SARS-CoV-2 virus doesn’t infect nerve cells in the nose. A new report in the journal Cell offers an explanation for how the virus shuts down our sense of smell and why it can take so long for some people to recover. The research shows that immune cells infiltrating nasal tissue in response to infection release compounds that disrupt the production of olfactory receptors—proteins on the surface of nerve cells that detect odor molecules. The researchers found that immune compounds interfere with the ability of parts of the genome to interact. These physical interactions in cell nuclei are essential for genes to direct the synthesis of proteins. The researchers also found that the downregulation of olfactory receptors persisted long after infection, which may explain why the sense of smell does not recover quickly in some people. The research was led by neuroscientist Stavros Lomvardas, a member of the Kavli Institute for Brain Science at Columbia University; Benjamin tenOever, a professor in the Departments of Medicine and Microbiology at NYU Langone Health; and Jonathan Overdevest, an assistant professor of Rhinology and Skull Base Surgery at the Columbia University Irving Medical Center. The findings may also help explain some of COVID-19’s other persistent neurological effects, including “brain fog,” headaches, and depression, according to the researchers.
A movement mystery solved
Eureka moments are a rarity in science. Instead, the discovery process is often slow and methodical. That is the case for a scientific collaboration between Alexandra Nelson, Anatol Kreitzer, and Louis Ptáček — researchers affiliated with the Kavli Institute for Fundamental Neuroscience at the University of California, San Francisco — that has endured for more than a decade. The researchers have been working to understand the cause of a movement disorder, known as PNKD (paroxysmal nonkinesigenic dyskinesia), which Ptáček successfully modelled in mice. People with PNKD experience attacks of involuntary movements after drinking alcohol or coffee, or in response to extreme stress. PNKD is rare but dyskinesias writ large are not, and there are few effective treatments. Through a series of experiments with PNKD mice, Nelson, Kreitzer, and their team worked out the nerve cell type and neural circuitry involved in the attacks and why the cells are misfiring. They homed in on a type of inhibitory neuron in the striatum, an area of the brain involved in the initiation of movement. In the PNKD model, the inhibitory cells were much less active than normal and could be manipulated to trigger the attacks. The activity level was sensitive to endogenous cannabinoids, cell signaling molecules that influence synaptic function and could be targeted for treatment. The findings were published in the Journal of Neuroscience.
How figure skates put dizziness on ice
According to Google, many of us want to know, “How do figure skaters not get dizzy?” That question trended during the recent Olympic Games and may do so again during the World Figure Skating Championships. To answer it, CNN turned to Johns Hopkins neuroscientist Kathleen Cullen, a member of the Kavli Neuroscience Discovery Institute. She studies how the brain processes motion and is an expert on the vestibular system, which senses head motion and helps us maintain our balance. (In a recent publication, for example, her team tested a longstanding theory about how the brain flexibly encodes information about voluntary movement.) Cullen told CNN how athletes have slowly trained their brains over time to avoid feeling dizzy after a spin: “There’s a really profound fundamental thing that happens in the brain of people like dancers or skaters over lots and lots of practice. And that’s basically a change in the way the brain is processing information.” The feeling of dizziness is the result of a difference between the brain and eye movement. Cullen suggested that skaters have learned to ignore the signals coming from the inner ear that suggest the body is still in motion, even once they’ve stopped spinning and their gaze is fixed.
Making memories last
At Rockefeller University's Kavli Neural Systems Institute, Robert Darnell studies the role of RNA-binding proteins in the mammalian brain. These proteins are important because they influence which genes are turned on or off during development and normal function. In a new study, Darnell and his team investigated the role of the RNA binding protein, FMRP, in pyramidal neurons, a cell type involved in learning and memory. FMRP is known to bind and regulate 900 different proteins, but it isn’t clear which ones it interacts with in pyramidal neurons and whether those proteins are the same in all parts of the cell.

Neurons are complex cells with different functional compartments, yet they are rarely studied that way. Pyramidal neurons have elaborate dendritic trees, with branches that form connections with other neurons. These connections, or synapses, are thought to be where memories are formed. They can be far from the cell body and rely on having the right ingredients nearby to make synaptic proteins, including RNA binding proteins such as FMRP. When the researchers dissected cells and look at FMRP’s interactions, they found it plays a dual role in the cells. In dendrites, FMRP binds to RNAs that control the expression of synaptic proteins, influencing how the cell responds to inputs; in the cell body, FMRP binds to RNAs that encode regulatory proteins that broadly affect gene expression. The findings suggest there is a feedback loop between the cell body and the dendrites that could help fine tune the synaptic changes that support learning and memory.